3 Minerals
Nahgeib Miller
Learning Objectives
After completing this chapter, you should be able to:
- Define a mineral.
- Explain how minerals are formed.
- Describe the common rock-forming minerals, including silicates.
- Identify minerals based on their physical properties.
With an appreciation for the origin of Earth and the global macroscopic processes of plate tectonics, we understand that the materials that make up the interior and the crust are essentially the same (they exist in different proportions, though). However, the materials that make up the mantle are molten and the materials that make up the crust are in a solid state. The transformation of mantle materials to solid rock occurs when the materials are exposed at the surface, during an eruption, and are added to a plate. The change in temperature results in a change of energy that allows for the combination of the mantle materials in a variety of solid forms. These solid forms are called minerals and it is these minerals that come together to make rock.
3.1 An Introduction to Minerals
Minerals are the “Lego blocks” used to build rocks. That is, rocks are made of and from minerals. Think of it this way: minerals are to rocks as students are to a class. Many different students can make up a class just like many different minerals make up a rock. A rock can also be made up of many crystals of the same mineral, just like a class can be made up of just one student (that must be a bad teacher!).
A more scientific definition of minerals is that they are characterized by:
- Solidity While minerals are chemical compounds, not all chemical compounds are minerals. Some chemical compounds exist in a liquid or gaseous state, while minerals must be in a solid state to be classified as such.
- Crystalline Structure This term is applied to particles or atoms that are arranged in a regular, repeating three-dimensional array. That is, the mineral that is visible to the eye is the result of millions of repeated three-dimensional stacks of the same microscopic mineral “block.”
- Ordered Internal Structure It is possible to have our solid microscopic mineral “block” stacked in a three-dimensional array with a chaotic pattern instead of a regular repeating one. Such a material is called a glass, not a mineral. A mineral must have an ordered arrangement of particles.
- Specific Chemical Composition The mineral building block that is arranged in a repeating three-dimensional array must also have a specific combination of atoms. Each unique mineral will have its own “recipe” of atomic combination ratios. For example, halite, or rock salt, has equal parts sodium (Na) and chlorine (Cl) to give NaCl.
- Inorganic Nature Any material derived from living tissue is not considered to be a mineral, with just one small catch. Organic material like coal and anthracite can be solid with a specific chemical composition; however, these materials are not crystalline and are therefore not considered to be minerals. Graphite, on the other hand, is crystalline and is considered a mineral even though it is derived from coal/anthracite. Technically, the requirement of an inorganic origin still holds because graphite is one step removed from living tissue.
- Natural Occurrence Minerals should occur in nature, which means that minerals created in a lab would not be considered as such. While artificial minerals would meet most of the criteria to be considered minerals, they would be of no use to geologists because no Earth processes would be recorded. That means we could not infer the crystallization environment, temperature of formation, weathering, erosion, transport mechanisms, or depositional environment.
3.2 A Brief Overview of Matter
In our previous example of halite as a mineral, sodium (Na) and chlorine (Cl) are elements that combined (reacted with) each other to create NaCl. The smallest part of an element that retains its physical and chemical properties is called an atom. An atom consists of a nucleus of protons and neutrons and a surrounding cloud of electrons that orbit the nucleus. Protons are positively charged, neutrons have no charge, and electrons are negatively charged. It is the electrostatic attraction between the protons in the nucleus and the electrons that is responsible for chemical reactions and bonding (Animation 3.1).
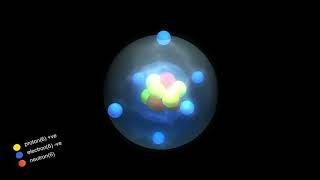
Click on the image to load the animation.
Usually, atoms of a specific element have the same number of neutrons as there are protons. However, there are small amounts of the same atom with different numbers of neutrons in the nucleus; these differing atoms are called isotopes. Let us take the element carbon as an example. Carbon generally has 6 protons and 6 neutrons in its nucleus, yielding an atomic number of 12 (in carbon-12, or C12). However, small amounts of carbon also exist, with 7 or 8 neutrons, yielding isotopes of carbon with atomic numbers 13 (C13) and 14 (C14), respectively.
When we think back to our definition of a mineral, the criterion of having a specific chemical composition speaks to the interaction of two or more atoms of elements in a certain fixed ratio or proportion. This interaction is called a chemical reaction. That is, for example, the combination of one sodium atom with one chlorine atom to make sodium chloride (NaCl). Chemical reactions form chemical compounds by either sharing electrons or transferring electrons from one atom to the other. These compounds are held together by the attraction between the positively charged nucleus (specifically, the protons in the nucleus) and the negatively charged electrons.
Chemical reactions in which electrons are transferred are a result of ionic bonding, as in the formation of NaCl (Figure 3.1).
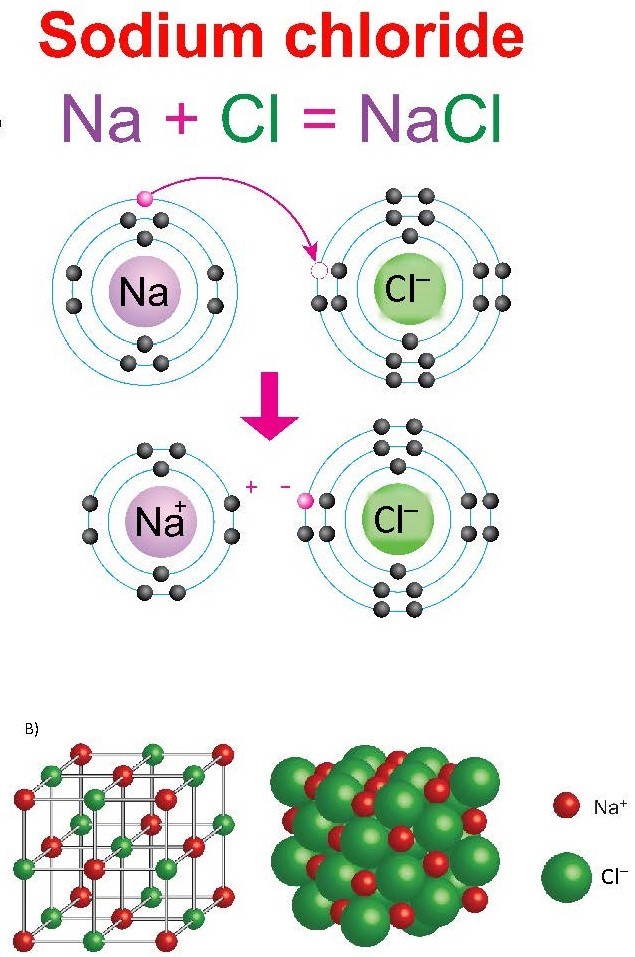
(a) Electron transfer in the NaCl formation reaction. (b) NaCl crystal structure
Chemical reactions in which electrons are shared are a result of covalent bonding, as in the formation of diamond (carbon; Figure 3.2).
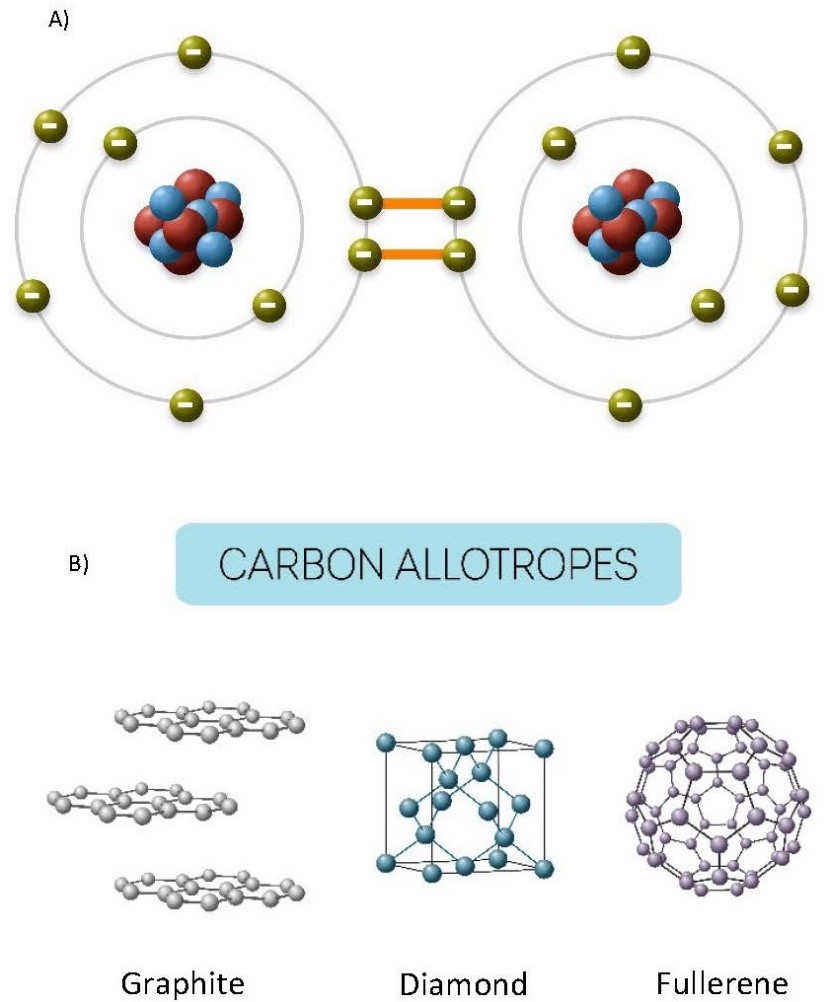
(a) Electron sharing in diamond. (b) Chemical structures (allotropes) of carbon
Covalent bonds are stronger than ionic bonding, manifesting in the mineral physical property of hardness, with covalently bonded minerals being harder than ionically bonded minerals. However, most minerals are ionically bonded.
Although we represent the atoms of elements in their stable form with an overall neutral charge (that is, an equal number of protons and electrons), in a chemical compound they actually exist as ions. Some atoms will want to lose an electron or two to become stable in the compound and some will want to gain an electron or two. When atoms give up electrons, they become positively charged overall (more protons than electrons) and are called cations. When atoms gain electrons, they become negatively charged overall (more electrons than protons) and are called anions. Anions and cations will combine based on their atomic size and charge. The larger an ion’s atomic charge, the more reactive the ion will be. Furthermore, the smaller the atomic size, the more reactive it will be (Figure 3.3). This is because the reaction or bonding between ions is due to the attraction between one ion’s nucleus and the other ion’s electrons. Therefore, the closer the nucleus can get to the other ion’s electrons, the stronger the potential for reaction and the stronger the bonds. Smaller atomic sizes allow the nucleus to get closer because the atomic radius is small. Hence the compound that would preferentially form would be between the smallest ions with the largest charges.
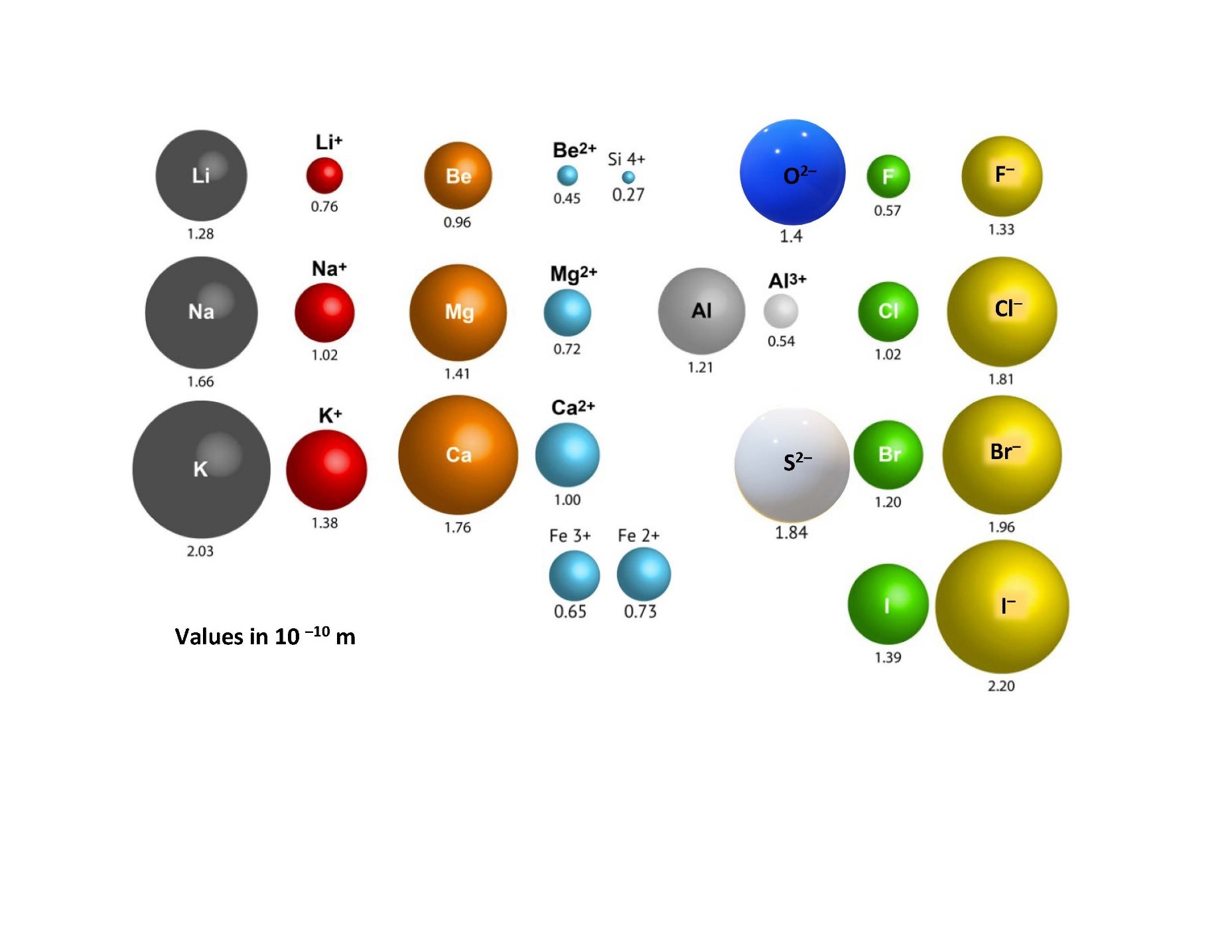
3.3 Mineral Formation
Minerals form in three ways:
- Crystallization from cooling molten rock As molten rock rises through Earth’s crust at spreading centres, convergences zones, and hotspots, it begins to cool and form minerals, which aggregate into rocks. Let us think of this molten rock as a “soup of potential” containing our common mineral-forming anions and cations. This “soup” is a high-energy environment that is about 1300 °C when the rock is completely molten; in this environment, the ions are not able to bond because they are vibrating too much. As the molten rock cools below 1300 °C, the roots of the first minerals start to form because the only ions that can bond are the ones that would make the strongest bonds. That is, they are able to form a stable bond in a high-energy environment. The smallest ions with the highest charge bond first. Referring to Figure 3.3, those ions would be silicon and oxygen, which would bond to form a larger silicate anion SiO4–4 (see Figure 3.4). This silicate anion forms the basis of 95% of all minerals in the crust.
- Crystallization from water evaporation As water percolates through Earth’s crust and flows over rocks exposed at the surface, it dissolves soluble minerals, returning their ions to another type of “soup of potential.” As the water evaporates, it can no longer hold the ions in solution: the ions bond to form minerals that then fall out as sediments. These sediments eventually accumulate and turn into rock.
- Recrystallization from existing minerals due to changes in temperature and pressure Existing minerals can be changed into new minerals without melting when they are exposed to new temperatures and pressures. Upon exposure to new prevailing temperatures and pressures, minerals will rearrange their crystal structure to achieve stability under these new conditions. The rearrangement results in the creation of a new mineral entirely.
3.4 Common Rock-Forming Mineral Classes
The crystal arrangement of minerals depends on how the anions are packed and how the cations are packed between the anions. This is because the anions are larger than the cations and will take up the most space. Minerals with similar anions will have a similar crystal structure and, therefore, similar physical properties, which vary slightly depending on the type of cations bonded with them. Hence it is advantageous to classify minerals based on their anions, yielding seven mineral classes, as follows:
- Silicates These contain silicon (S) and oxygen (O) bonded in silicate tetrahedra (SiO4–4).
- Carbonates These contain carbon (C) and oxygen (O) bonded in a carbonate anion (CO3–).
- Oxides These result when an oxygen (O–2) anion bonds with a metallic cation.
- Sulfides These contain a sulfur (S–) anion bonded with a metallic cation.
- Sulfates In these minerals, cations are bonded with a sulfate anion (SO42–), which contains sulfur and oxygen.
- Halides These minerals have cations bonded with bromide (Br–), chloride (Cl–), fluoride (F–), and iodide (I–) anions.
- Hydroxides These minerals have cations bonded with a hydroxyl anion (OH–), which contains oxygen (O) and hydrogen (H).
Because the vast majority of rock-forming minerals are silicates (Figure 3.4), we will focus on this mineral class.
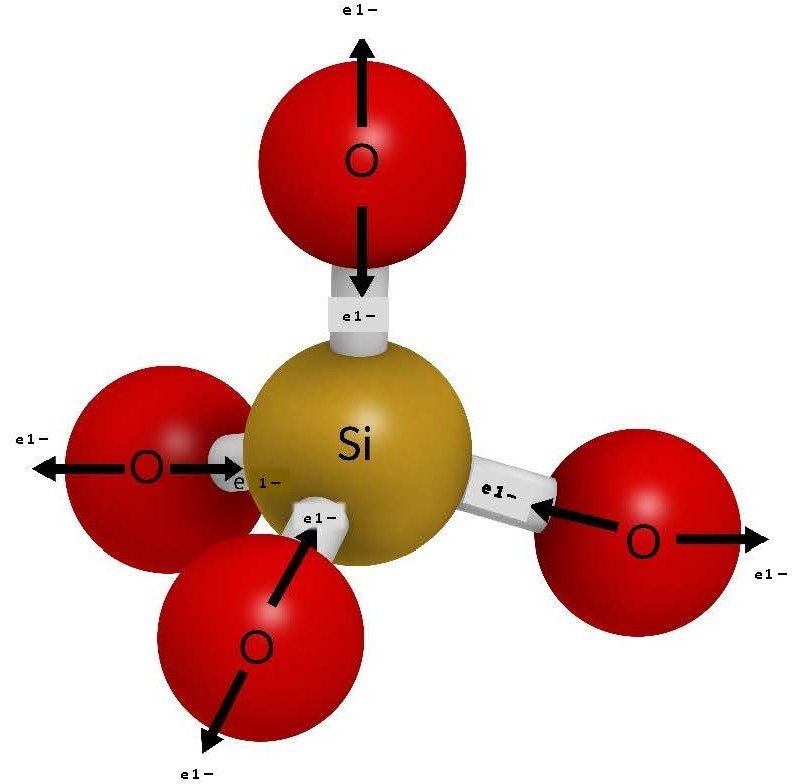
Note that each oxygen anion contributes one electron each to the silicon, leaving one electron each for bonding. Therefore, the overall charge of the molecule is 4¯
Silicates
The silicates mineral class is based on the arrangement and packing of the silicate anion as the “soup of potential” cools. As previously stated, the first thing that forms in our high-energy soup of potential is the silicate anion, which then bonds with any available cations. Due to the high-energy environment, the only cations capable of bonding with the silicate anion are those that are small with high charges, such as iron (Fe3+, Fe2+), magnesium (Mg2+), and aluminum (Al3+). The name of a mineral in each silicate group depends on the ratio of the bonded cations, where a mineral with 100% iron will be at one end of the group and a mineral with 100% magnesium will be at the other end. Note that most (if not all) of the silicon (the smallest cation with the biggest charge) is used up to create the silicate anion, which has an overall 4– charge due to the available electron each oxygen, bonded to the silicon, provides.
As the soup cools the arrangement of the silicate anions are in order as follows:
- Isolated Tetrahedra Isolated tetrahedra form the olivine group of silicate minerals. As the soup of potential continues to cool, after the formation of the silicate tetrahedra, the Fe3+, Fe2+, and Mg2+ cations are able to bond due to the reduction in energy of the environment. Therefore, all of the available electrons provided by the oxygens in the tetrahedron are bonded to a cation, which is in turn bonded to another oxygen in an adjacent tetrahedron (Figure 3.5a). Each tetrahedron is effectively cut off or isolated from the others because they are separated by a cation. This also creates one cleavage plane in the crystal structure (we will discuss this in the next section). The minerals in this group will fall somewhere between forsterite (100% Mg as the cation; Figure 3.5b) and fayalite (100% Fe as the cation). This variation in the composition of the mineral yields the chemical formula (Mg, Fe)2SiO4.
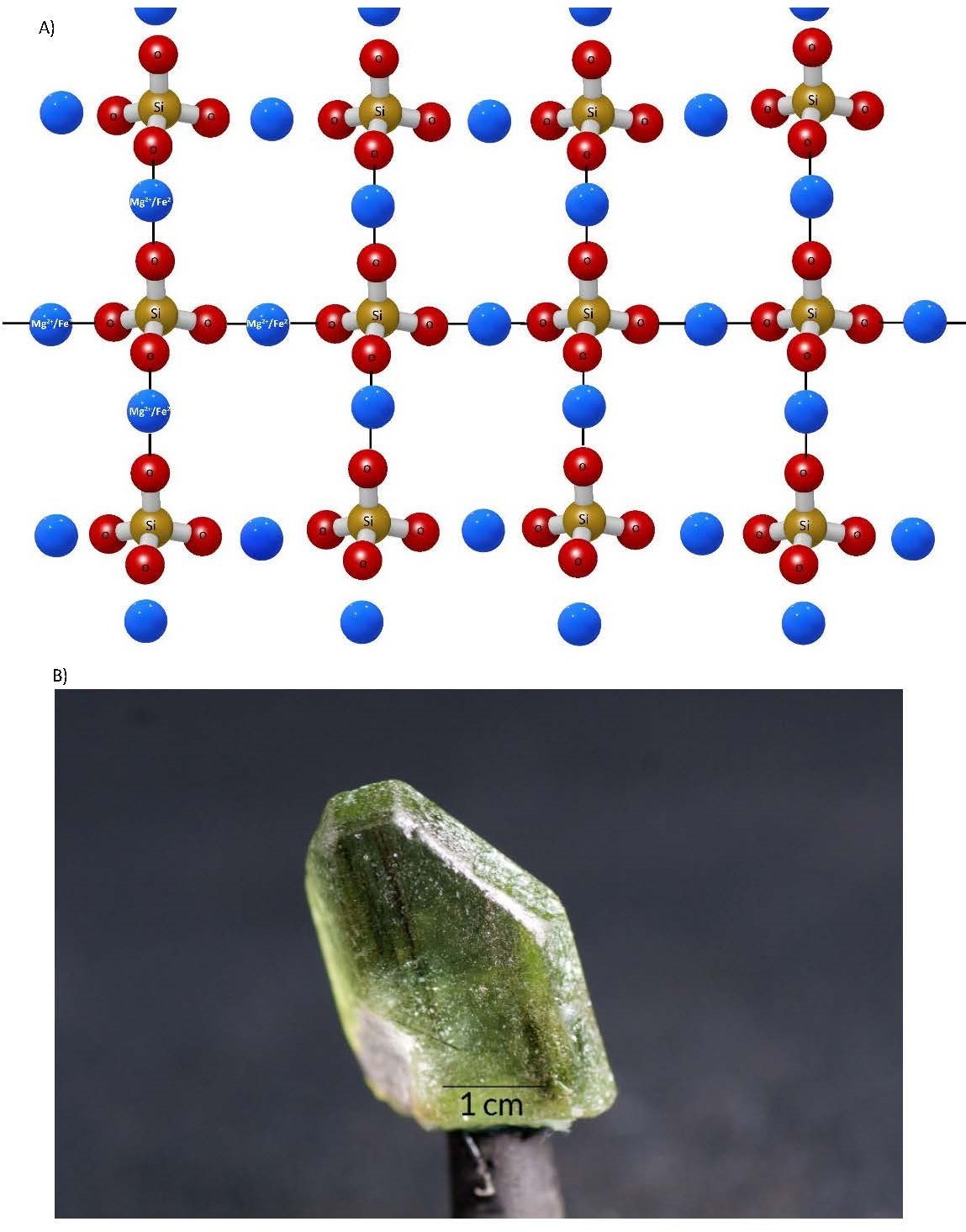
(a) 3D illustration of isolated tetrahedron silicate mineral. (b) Forsterite, a mineral in the olivine group
- Single–Chain Tetrahedra Single-chain tetrahedra form the pyroxene group of silicate minerals. As cooling continues, the reduction of energy in the environment allows for adjacent tetrahedra to bond through a common oxygen. That is, one oxygen in a tetrahedron is bonded to the silicon in another tetrahedron, with the other three oxygens bonded to a cation. This creates a repeating link between adjacent tetrahedra, like a single chain — hence the name. The crystal structure that eventually results creates two cleavage planes at 90° to each other. A wide range of cation substitutions can occur, yielding a wide range of minerals, of which augite and diopside are the most common. The chemical formula for the pyroxene group is (Mg, Fe, Ca, Al)SiO3 (Figure 3.6). Note that aluminum can substitute for the silicon.
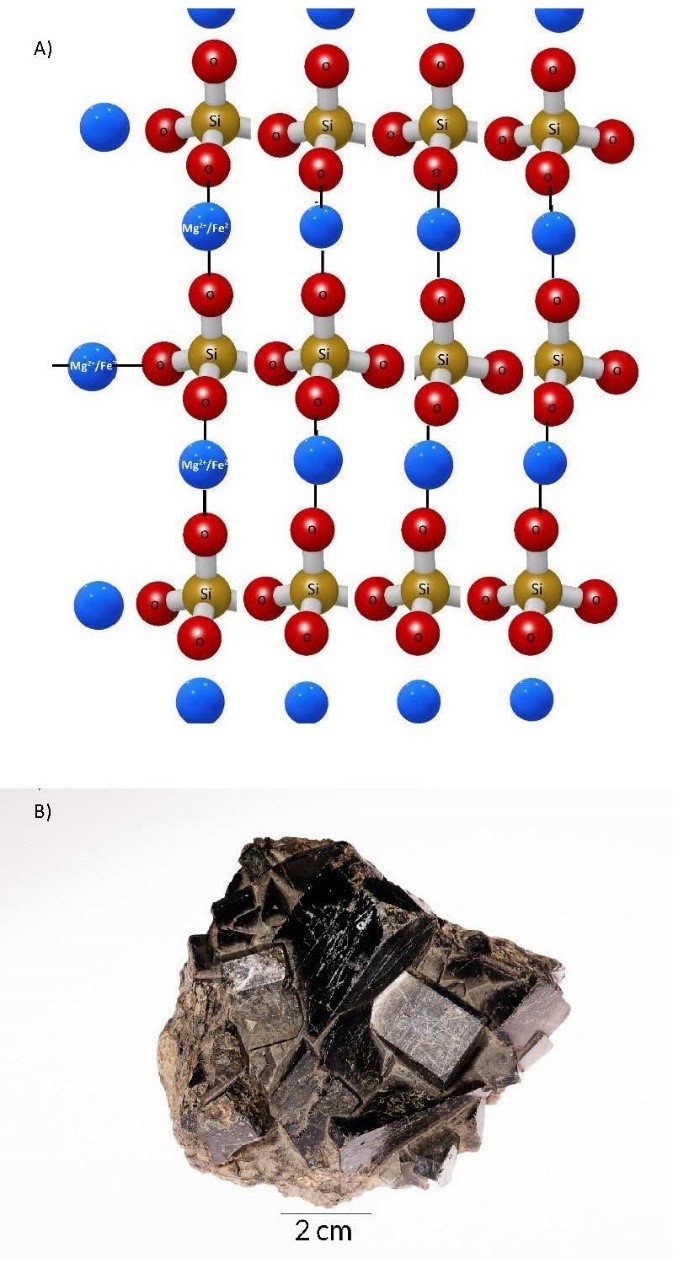
(a) 3D illustration of single-chain tetrahedron silicate mineral. (b) Augite, a mineral in the pyroxene group
- Double–Chain Tetrahedra Double-chain tetrahedra form the amphibole group of silicate minerals. As cooling continues below the temperature at which pyroxenes form, the lowered energy allows two of the oxygens in adjacent silicate tetrahedra to share the same silicon ion. This leaves only the other two oxygens to bond with a cation. The lowered energy allows for larger cations to bond in a more complex crystal structure that has two single chains stitched together to form a double chain. This crystal structure yields two cleavage planes, at 56°/124° to each other. A wide range of cation substitution yields numerous mineral names within the group, as reflected by the chemical formula (Na, Ca)2(Mg, Fe)5Si8O22(OH)2 (Figure 3.7).
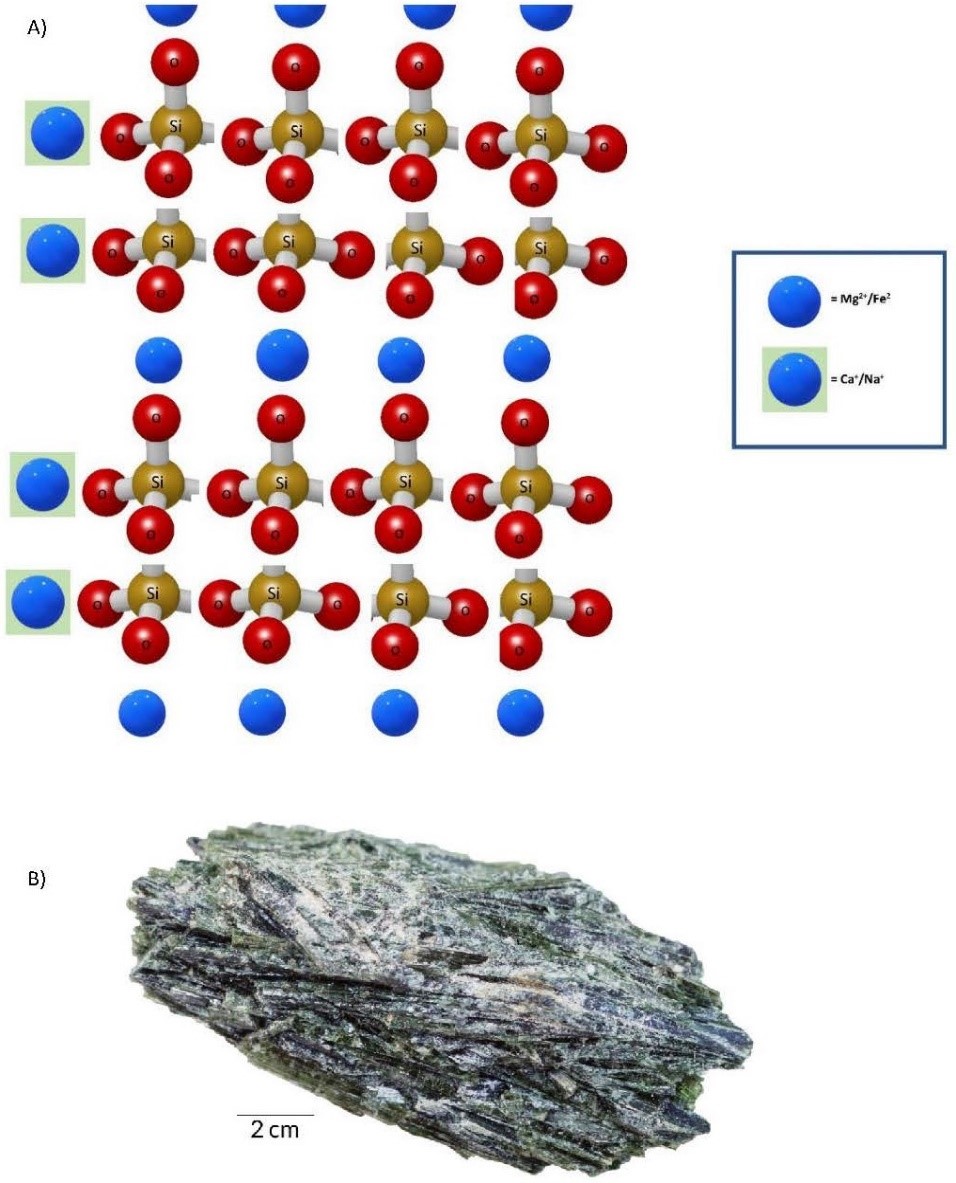
(a) 3D illustration of a double-chain tetrahedron silicate mineral. (b) Actinolite, a mineral in the amphibole group
- Sheet Tetrahedra Sheet tetrahedra form the mica group of minerals. Cooling of molten rock in our “soup of potential” to below that at which amphiboles form allows three of the four oxygens to bond with another silicon in an adjacent tetrahedron. Essentially, these are the three oxygens at the base of the tetrahedron, resulting in a sheetlike crystal structure (Figure 3.8). The lower temperature also allows larger cations to bond, yielding two types of chemical compositions: muscovite, KAl2(AlSi3O10)(OH)2, and biotite, K(Mg, Fe)3(AlSi3O10)(OH)2. The sheetlike crystal structure yields one perfect cleavage plane.
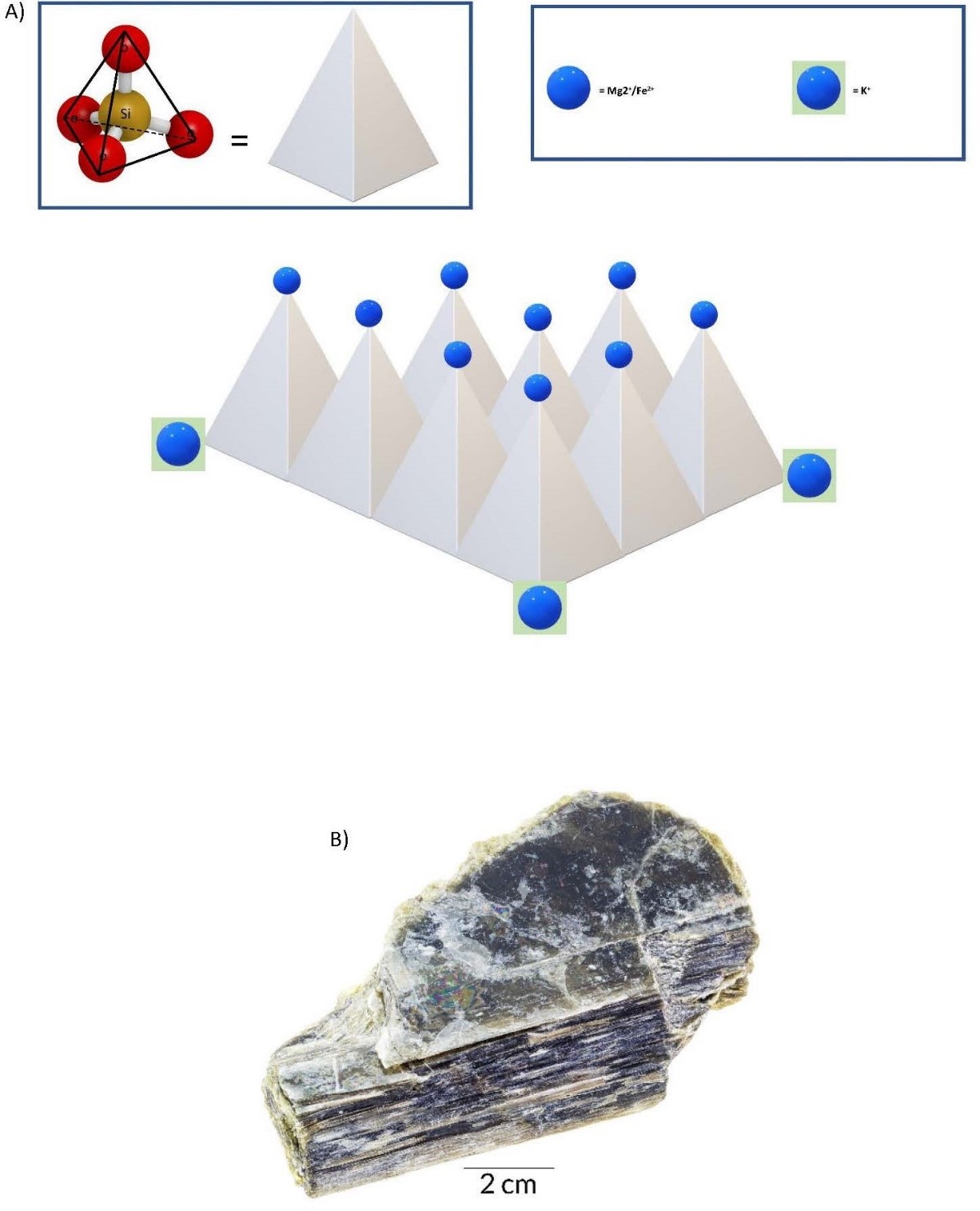
(a) 3D illustration of a sheet tetrahedron silicate mineral. (b) Biotite, a mineral from the mica group
- Framework Tetrahedra Framework tetrahedra form the feldspar group of minerals, which occur toward the end of the cooling phase, before complete solidification. In this lower-energy environment, most of the oxygens are bonded to a silicon in an adjacent tetrahedron with a few cation bonds. The cleavage planes would be arranged at 90° to each other along the cation bonds. The large, weakly charged cations of K, Ca, and Na now bond easily, yielding two types of chemical compositions. These are orthoclase feldspar, KAlSi3O8, and plagioclase feldspar, (Ca, Na) AlSi3O8. The cleavage planes are defined by the cation bonds and are arranged at 90° to each other. The last mineral to crystallize is pure silica, with no cation bonds, in which state it is called quartz. Because there are no cations, there are no natural planes of weakness, and thus quartz has no cleavage.
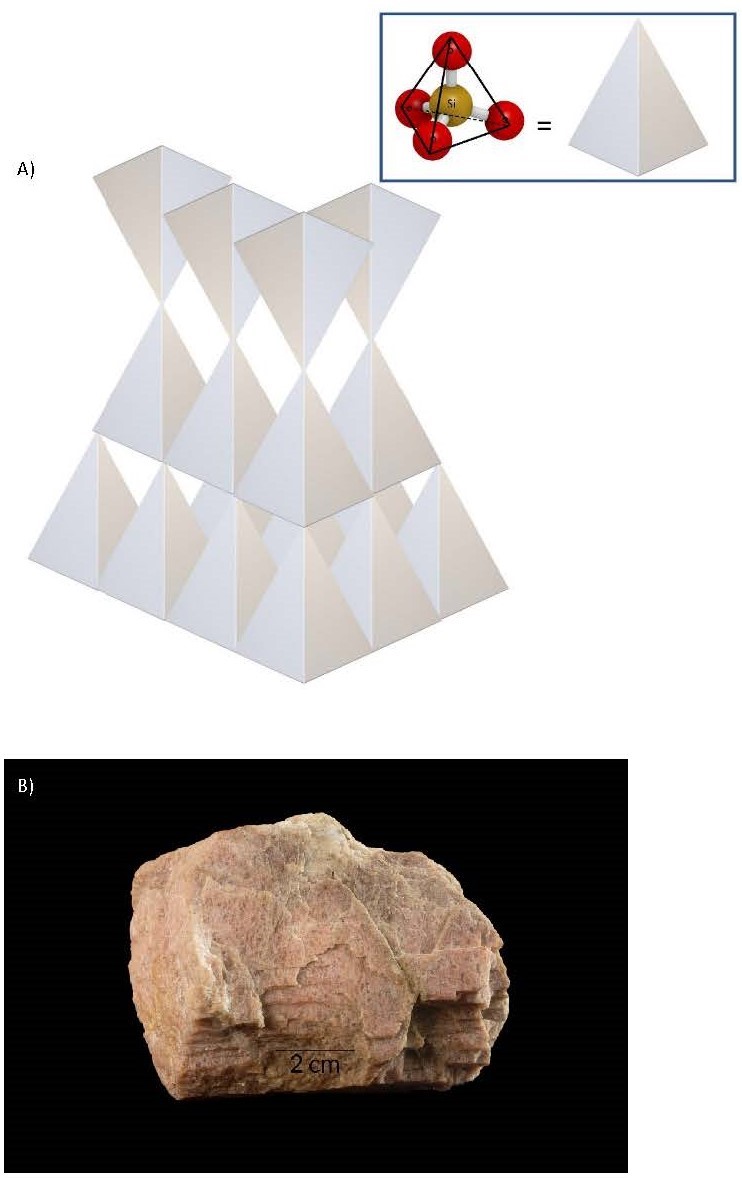
(a) 3D illustration of a framework tetrahedra silicate mineral. (b) Orthoclase, a mineral from the feldspar group
3.5 Mineral Identification Using Physical Properties
The chemical properties and crystal structure of a minerals are macroscopically expressed in its physical properties. These properties — colour, lustre, streak, hardness, cleavage, fracture, density, and crystal habit — can be used to differentiate between the main mineral groups, including the silicates. Let us first explore the individual mineral physical properties in order to understand how to use these to identify minerals.
The Physical Properties of Minerals
- Colour The colour of a mineral is strongly influenced by its cations, with metallic cations creating deeper, darker colours than non-metallic cations.
- Lustre Lustre describes how the surface of a mineral reflects light. Not to be confused with colour, which is an internal expression of reflected light, lustre is a surface expression of how incident light bounces off the mineral. Lustre can be described as glassy/vitreous, or reflecting light like a glass (even if it has a dark colour!); dull, or without much reflection; metallic, or reflecting like a metal; greasy, or reflecting light like an oily surface would; or iridescent, or reflects light like the surface of a pearl. Ionically bonded minerals tend to be glassy, while covalently bonded minerals tend to have a more variable lustre.
- Streak Streak is the colour of the powdered form of a mineral, which is more representative. However, this state can be achieved only for minerals that are softer than the streak plate.
- Hardness Hardness is a measure of how resistant a mineral is to being scratched. The stronger the prevailing chemical bonds within the crystal structure, the harder the mineral. That means that ionically bonded minerals have a lower hardness than covalently bonded ones. Also, quartz will be harder than olivines because tetrahedron–tetrahedron bonds are stronger than tetrahedron–cation bonds. Minerals are identified with a hardness according to the relative scale of Moh’s Scale of Hardness.
Table 3.1. Moh’s Scale of Hardness
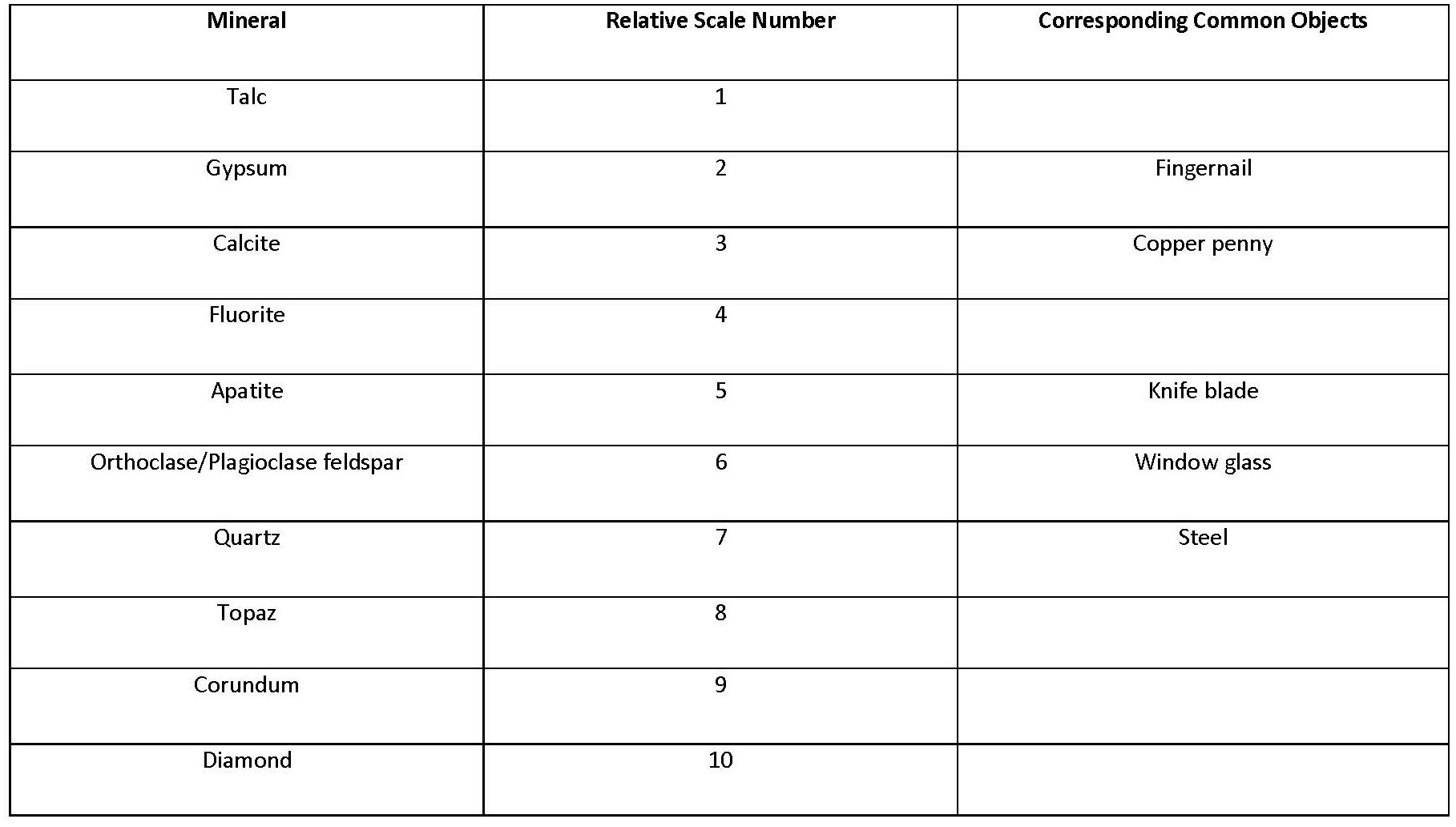
- Cleavage Cleavage is the tendency for a mineral to break along planar surfaces and is an expression of internal chemical bonds. Ionically bonded minerals tend to be weaker than covalently bonded minerals and therefore produce better cleavage planes, because weaker bonds are easier to break under an incident force. Furthermore, cation–tetrahedron bonds (olivines, pyroxenes, amphiboles, and micas) are weaker than tetrahedron–tetrahedron bonds (quartz) and tend to form better cleavage planes. The number of planes and the angles those planes make with each other depend on the crystal structure and chemical bonds and can be used to differentiate between minerals that have similar colour and hardness (Video 3.1).
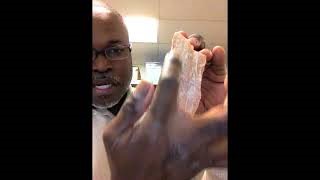
- Fracture The term fracture describes the tendency for a mineral to break along irregular surfaces and is also an expression of internal chemical bonds. Essentially, fracture is the opposite of cleavage because minerals that have good cleavage will not have many (or any) fracture surfaces. Another way to look at fracture is that tetrahedron–tetrahedron bonds (quartz) are stronger than cation–tetrahedron bonds (olivines, pyroxenes, amphiboles, and micas) and tend to form irregular fracture surfaces rather than cleavage planes (Video 3.2).
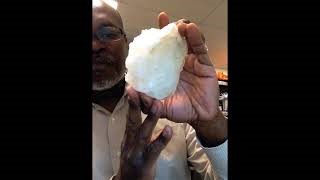
- Density A mineral’s density is a direct manifestation of the packing of the ions in the crystal structure as well as the atomic mass. Abundant smaller, heavier metallic cations will create a denser mineral than with larger, less massive ions. That is, minerals with higher metallic content, such as olivines, pyroxenes and amphiboles, are denser than minerals with low metallic content, such as quartz.
- Crystal Habit A mineral’s crystal habit is the geometric description of the overall shape of the completely developed mineral. A mineral’s crystal habit depends on the internal crystal structure that grows in a repeating three-dimensional array, along with the speed and prevailing direction of crystal growth (see Table 3.2).
Although the physical properties of hardness, cleavage, and colour are the most useful in differentiating between minerals, the use of all eight properties allows us to accurately identify minerals in the different silicate groups as well as minerals in the general mineral classes. Furthermore, a definitive identification for the mineral calcite is its effervescent reaction with dilute HCl (Video 3.3).
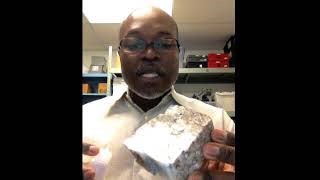
Table 3.2 Common minerals and their physical properties. A Density of 1–3 g/cm3 is considered low; 3.1–4 g/cm3 is considered medium density; above 4 g/cm3 is considered high density.

A naturally occurring solid, inorganic, three-dimensional array of specific chemical composition with an ordered crystalline structure
A three-dimensional array of elements with a chaotic pattern
The smallest part of an element that retains its physical and chemical properties
The central, high-density portion of an atom consisting of protons and neutrons
Positively charged particles in an atom’s nucleus
Noncharged particles in an atom’s nucleus
Negatively charged particles that orbit an atom’s nucleus and that take part in atomic reactions
Atoms of an element with the same number of protons but a different number of neutrons
The interaction of two or more atoms of elements in a certain fixed ratio or proportion
A transfer of electrons from one atom to another
The sharing of electrons between atoms
An atom or molecule that has gained or lost an electron or electrons
Ions that have resulted from an atom giving up an electron or electrons and that are thus positively charged
Ions that have resulted from an atom gaining an electron or electrons and that are thus negatively charged
Minerals with a silicate anion
A silicate structure in which a silicate anion is separated from an adjacent anion by a cation
Silicates in which oxygens of adjacent tetrahedra bond to a shared cation
Silicates in which one oxygen in a tetrahedron bonds with the silicon of an adjacent tetrahedron
Silicates in which one oxygen in a tetrahedron bonds with the silicon of an adjacent tetrahedron
Silicates in which two oxygens in a tetrahedron bond with the silicon of an adjacent tetrahedron
Silicates in which two oxygens in a tetrahedron bond with the silicon of an adjacent tetrahedron
Silicates in which three oxygens bond with the silicon of an adjacent tetrahedron
Silicates in which three oxygens bond with the silicon of an adjacent tetrahedron
Silicates with few cation bonds
Silicates with few cation bonds
Solid silica with no cation bonds
A description of how the surface of a mineral reflects light
The colour of the powdered form of a mineral
A measure of how resistant a mineral is to being scratched
A range from 1 to 10 defining the relative degree to which a mineral resists being scratched
The tendency for a mineral to break along planar surfaces
The tendency for a mineral to break along irregular surfaces
The geometric description of the overall shape of a completely developed mineral